The first image taken of a black hole, the picture that finally turned artists’ impressions into a reality, was of the supermassive black hole at the centre of the galaxy Messier 87.
Most supermassive black holes are found in the centres of galaxies. They sit in the gravitational driving seat as hundreds of billions of stars in the surrounding systems happily orbit them, just like the planets orbit the Sun at the centre of our Solar System.
The black hole at the centre of Messier 87 lies at the more massive end of the supermassive scale, cramming a mass that’s six billion times that of the Sun (six billion solar masses) into an area the size of Neptune’s orbit.
But as huge as that might sound (especially when compared to the black hole at the centre of the Milky Way, which is a mere four million solar masses), it’s by no means the most massive black hole that we know of.
That title goes to TON 618, which is an astonishing 66 billion solar masses. It’s so big that astronomers had to invent a new term to describe it; hence, TON 618 is what’s become known as an ultramassive black hole.
To give you an idea of just how mind-bogglingly big TON 618 is, imagine taking all the stars in the Milky Way and squishing the matter in them down to create a black hole. Even if you did that, you would still be a few billion Suns’ worth of matter shy. So how did TON 618 become such a behemoth?
What would really happen if you got near a black hole
Black holes are made of vast amounts of matter that have accumulated in one spot and been packed together as densely as possible, to the point where the gravitational pull from the accumulation is so strong that not even light can escape it.
But that doesn’t mean that a black hole is a giant monster with an insatiable appetite, roaming the Universe and devouring everything it encounters.
TON 618, the most massive black hole we know of, still has an entire galaxy of stars happily orbiting it and not getting sucked in. It’s a common misconception that black holes act like vacuum cleaners, when in reality it’s incredibly difficult to grow a black hole.
Read more:
For a start, you have to get material close enough for it to be affected by the black hole’s gravity. And when you consider how dense black holes are, due to all that matter being squashed into such a tiny space, and how vast the Universe really is, you start to realise just how difficult that can be.
If matter does stray too close, however, it will get pulled in by the black hole’s gravity and that gravity increases dramatically the closer you get. Think of it like walking up a hill, but the hill becomes steeper with every step you take. It’s brutal.
The gravity around a black hole increases so quickly that if you were to approach one with your feet first, the pull of gravity at your feet would be so much stronger than at your head that you’d get stretched out like spaghetti. (In perhaps the best example of jargon the science world has to offer, the process is legitimately referred to as ‘spaghettification’.)

We see this happening to stars and clouds of gas as they get close to a black hole. Once spaghettified, the matter then gets pulled into orbit around the black hole and flattened out into a swirling disc of material.
The process is similar to the way you create a pizza base by tossing the dough above your head – as it spins, the dough gradually flattens out. Once it’s in that spinning disc, the matter (usually hydrogen gas as this is the most common element in the Universe) will settle into a nice, happy orbit around the black hole, far away from the point of no return: the event horizon.
The event horizon is the sphere around the black hole that we use to ascertain its size; the larger the event horizon, the more massive the black hole.
While the matter inside the event horizon is being crushed down into an infinitesimally small space, known as the singularity, the size of a black hole is taken to be the region of space from which we no longer receive any light.
It’s the distance away from the singularity where you’d have to be travelling faster than the speed of light to escape the black hole’s gravity. If you cross the event horizon, you’re done for, officially part of the black hole and contributing to its total mass forevermore.
But getting matter to cross the event horizon is what’s so difficult about growing black holes. How do you move material out of a happy, stable orbit around the black hole and get it to ‘fall in’, helping the black hole swell to massive proportions?
The Sun and Earth provide a good demonstration of the difficulty. Earth is in a stable orbit around the Sun and despite the star’s huge size and far greater gravity compared to our planet, it’s thankfully unable to pull us in towards it.
What actually falls into a black hole
The reason material is able to cross the event horizon is collisions between particles in the disc of material orbiting it. Atoms of hydrogen gas collide and transfer energy, just like balls on a snooker table.
If you’re a talented snooker player you might be able to hit the cue ball against a coloured ball and get the cue ball to stop dead, transferring all of its energy to the coloured ball, which shoots off across the table.
A similar exchange in energy can happen to colliding particles in the disc of material orbiting a black hole; the particle that gains all the energy will get a boost, pushing it further away from the black hole.
But the particle that loses its energy will have nothing to resist the gravity pulling on it, and gradually slide down to cross the event horizon and become part of the black hole.
Read more:
- Could we harness energy from a black hole?
- New perspective of supermassive black hole confirms 30-year-old prediction
Not every collision will result in such a drastic energy exchange; on average there’ll be an equalling out of the energy. But eventually, one particle will have enough collisions and be unlucky enough to lose energy in every one, bringing it ever closer to the black hole.
It’s only when the particle has a collision in the region known as the ‘innermost stable circular orbit’ (ISCO) that it will finally cross the event horizon to become a part of the black hole.
The ISCO doesn’t exist in Newton’s theory of gravity, which we all learn at school. Newton’s theory says that all perfectly circular orbits, no matter their distance, are very stable.
That means if an object on a circular orbit is unsettled slightly, it’ll remain largely on the same orbital path. For instance, if something was somehow orbiting near the Sun in a perfect circle and was nudged by another object, its orbit might become more oval, but it would continue orbiting the Sun.
That’s not the case in Einstein’s theory of relativity, however. As you get closer to an object like a black hole, there comes a point where, if you nudge an object on a circular orbit – or, say, one particle were to collide with another – that object couldn’t correct its path and would end up spiralling in towards the black hole.
That point is the ISCO. It lies around three times further out than the event horizon and, usually, marks the inner edge of the orbiting disc of matter. This process of particles colliding, exchanging energy and falling over the event horizon – accretion – takes a very long time.
We’re talking millions of years just to get a black hole to a size where it can snack on a passing gas cloud that drifts too close.
How much matter can fall into a black hole?
Complicating matters even further is the fact that there’s also a limit to how much matter a black hole can accrete in a given time. The Universe effectively puts a black hole on a diet if it becomes too gluttonous.
This happens because the disc of matter orbiting a black hole is moving and colliding at such an incredible speed, that it starts to heat up due to friction. It’s heated to such an extreme temperature that it glows like metal in a forge.
The good news about this glow is that it allows us to spot black holes and measure how massive they are. The light can, and does, outshine entire galaxies, making them look like single pinpricks of light.
These pinpricks were originally dubbed quasars, a portmanteau of quasi-stellar, because they look like stars but aren’t stars. (It was only after the Hubble Space Telescope launched in the early 1990s that astronomers could make out the galaxy of stars that went with each quasar and their true role in growing supermassive black holes was revealed.)
The bad news for black holes is that the glow is so intense that it exerts a pressure outwards (radiation pressure) that prevents any more matter from falling in.
If there’s too much material in the disc, the radiation pressure increases and pushes material away from the black hole.
There’s a fine balance between the size of the black hole and the quantity of matter in its disc, and when a certain threshold is reached (depending on how massive the black hole is) the rate at which it can accrete material is capped.
How big can black holes get?
What makes TON 618 so exceptional is the sheer amount of physics standing in the way of a black hole growing to that size. But while we’ve known for a long time that radiation pressure places a limit on how fast a black hole can grow (known as the Eddington limit, after astronomer Sir Arthur Eddington), what we didn’t know until recently was whether there’s a limit to how massive a black hole can become.
As far as we know, there isn’t. Theoretically, you could keep on merging black holes to your heart’s content, gradually increasing the overall size, as if you were making a giant snowball. But there is a limit to how big black holes can grow via accretion.
In 2015, Prof Andrew King at the University of Leicester published a paper that pointed out a quirk of gravity around black holes. The quirk allowed him to estimate the maximum mass a black hole could achieve via accretion: 50 billion solar masses (but it could be 270 billion solar masses if the black hole was spinning fast in the same direction as its galaxy).

At the root of this quirk is the position of the ISCO, which, like the event horizon, is related to how massive the black hole is. As the black hole’s mass increases, the ISCO gets pushed further out.
The problem for a growing black hole is when the ISCO is pushed beyond what’s known as the ‘self-gravitational radius’. The distance that the self-gravitational radius sits around a black hole is another factor that’s related to the mass of the black hole, but it also depends on the object that’s approaching it.
The self-gravitational radius marks the point at which the pull of gravity holding the approaching object together (self-gravity) is stronger than the pull from the black hole.
The self-gravitational radius is why we’re all here. It’s why we’re able to have entire galaxies surrounding supermassive black holes; beyond this radius, gas in a galaxy is attracted more to itself than to the black hole lying at its centre.
If this wasn’t the case, gas would never collapse to form stars and instead our atoms would just be part of one giant disc of material orbiting a supermassive black hole.
It was King who pointed out that when a black hole reaches 50 billion solar masses, the ISCO gets pushed beyond the self-gravitational radius. What that means is that, no matter how many collisions they have, any particles in the disc of material orbiting the black hole will never lose enough energy to reduce their orbit to a point that allows them to reach the ISCO and spiral into the black hole.
Instead, the pull of gravity from all the other particles in the disc will always be stronger than the pull from the black hole. That’s if there even is a disc of material around the black hole – because any matter that isn’t on a direct, ‘bullseye’ trajectory with the singularity as it approaches, won’t get pulled into a disc. Instead it will loop around the black hole, spaghettified, but otherwise unscathed.
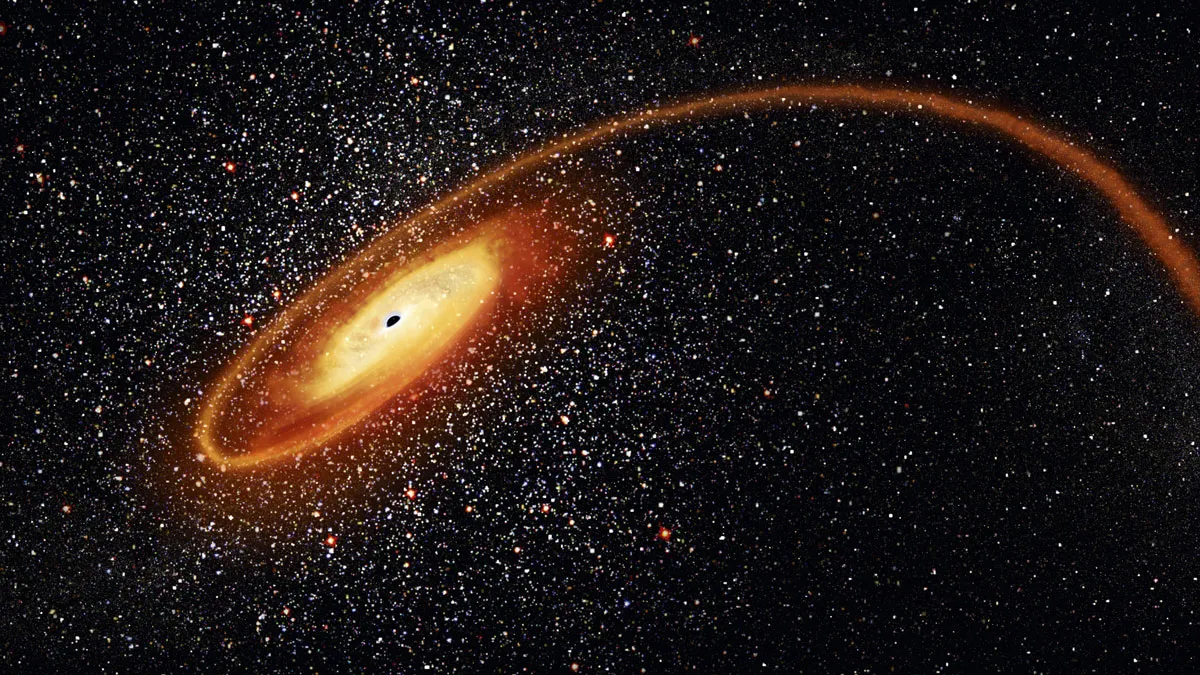
This lack of a disc means that we also won’t be able to spot such a black hole, as there’ll be no luminous matter around it lighting up like a Christmas tree.
This is why TON 618 is so interesting to astronomers: with an ultramassive black hole of 66 billion solar masses, it lies above King’s estimate of the maximum limit (50 billion solar masses) for a non-spinning black hole.
Most black holes are spinning, which increases the mass they can reach before they push the ISCO beyond the self-gravitational radius, but it still means that TON 618 could be nearing its maximum possible mass.
Past their prime
It’s fascinating to consider the implications of all this. We could be approaching the point in the Universe’s history in which black holes start reaching their limit.
If black holes stop accreting and growing in this way, then they’ll also stop glowing. Quasars will begin to wink out as black holes age past their glory days.
It’s possible that there are other black holes in the Universe that have already reached ultramassive proportions.
We can try hunting for them, but without the glow of a disc orbiting around them, we’ll never spot them. There could even be ultramassive black holes hiding right under our noses…
Read more: